LIDAR operates on the same principles as RADAR except that it uses light rather than radio waves to collect information. There are three generic types of LIDAR:
Range finders are the simplest of the LIDARs and are used to determine the distance to a solid or hard target. When mounted in an aircraft and coupled with imaging instrumentation and a GPS, these instruments can provide 3-D topographic maps.
DIAL is used to measure chemical concentrations in the atmosphere (open air). A DIAL LIDAR uses a laser wavelength that is strongly absorbed by the target compound and a second nearby wavelength that is not absorbed by the target compound. The difference in intensity of the two return-signals can be used to calculate the concentration of the compound being investigated.
Doppler LIDAR is used to measure the velocity of a moving target. When light transmitted from the LIDAR hits a moving target, the wavelength of the light reflected/scattered off the target changes slightly. This is known as a Doppler shift. This type of LIDAR finds uses in speed limit enforcement and weather predictions (cloud and wind speed as well as density). A complete treatment of LIDAR can be found in Argall and Sica 2002.
LIDARs consist of a source transmitter, receiver, and detector system. All uses of LIDAR involve laser light operated in the UV, visible, or infrared wave range that is transmitted toward a target. The light interacts with the target where it is either absorbed or reflected/scattered back to a measuring device. They can be deployed in monostatic or bistatic configurations. The monostatic configuration is employed the most in contaminant profiling. The system can be set up in either a coaxial configuration where the laser beam is transmitted within the receiver's field of vision or in a biaxial fashion where the transmitting and receiving units are adjacent to each other (Exhibit 1).
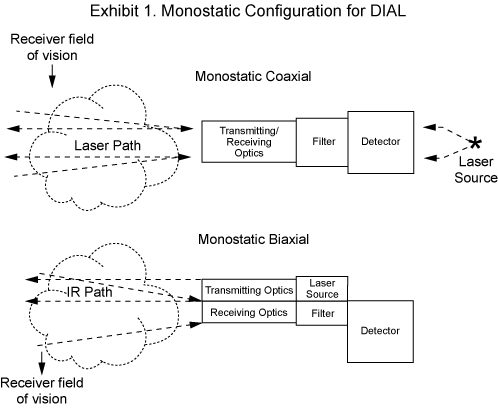
In the bistatic configuration, which is seldom used, the light source and the detector are at some distance apart (Exhibit 2). The receiving optics gather reflected light from the source beam and direct it through a filter to the detector. The bistatic system can only detect light from a small layer of the atmosphere at a time and the detector must be frequently moved to obtain a contaminant profile (Argall and Sica 2002).
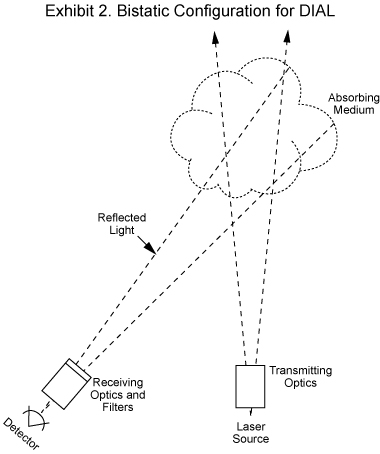
Source
LIDARs use lasers for their source and hence do not require a sending telescope as do Fourier Transform Infrared (FTIR) spectroscopy and Ultraviolet Differential Optical Absorption Spectroscopy (UV-DOAS); however, they generally require a mechanism for widening the laser beam to make it "eye safe." For the DIAL system, an appropriate wavelength is chosen for the species to be measured along with a nearby wavelength that will not be absorbed by the target compound. The laser can be transmitted as a continuous-wave or pulsed source. Continuous waves are used when the signal is integrated over a long time period or when the target is close. Pulsed waves are at a much higher energy level than can be maintained during a continuous emission, and they are used for long-range remote sensing or when the signal integration time needs to be short. Tunable laser systems that allow for quickly changing wavelengths are available to measure a wider range of contaminants.
Receiver
The receiver may have a Cassegrain or Newtonian telescope or a single fixed condensing lens that focuses the returning or transmitted light (depending upon system deployment). Before being directed to the detector, the backscatter light is usually processed to filter out background.
Detector
LIDAR detectors convert the returning light into electrical currents, which are then processed into photocounts. Photomultiplier tubes have been employed the most in DIAL systems, but semiconductor devices, such as mercury-cadmium-telluride (MCT) detectors and avalanche photodiodes are finding increasing use. To function properly MCT detectors have to be cooled to liquid nitrogen temperatures.
Output
Unlike FTIR or UV-DOAS, DIAL produces a measure of discrete concentrations versus distance along the line of the beam path. This allows for the values to be superimposed on a map or other visual aid to show where low and high point concentrations are occurring. A series of closely placed measurements over a site can be plotted to show an estimate of the spatial distribution and quantity of a chemical (e.g., an isopleths map).
Chemical Detection
LIDAR has historically been used in the environmental field for measuring criteria pollutants in the upper atmosphere. As the equipment becomes more portable and less expensive, it is gaining acceptance for industrial and commercial applications. The proper detection and identification of a chemical species using DIAL is directly dependent upon it having a unique absorption frequency over the path being measured (i.e., there are no other chemicals present that have the same absorption frequency) and the availability of a laser source that emits that frequency. In the past, the lack of laser sources that emit specific bands has limited the number of chemicals that this technology can identify; however, as more reliable tunable lasers become available the list of detectable chemicals should increase. In addition to specific chemical detection, a DIAL system can be used in a more "open" mode much like a point source organic vapor analyzer. In this mode, a chemical family, such as alkanes, is measured by picking a band that is common to many and interpreting the results as an "average." The Alberta Research Council study given below is an example of this use.
Detection limits are largely dependent on the path distance, atmospheric conditions, and the instrument being used. However, an examination of the literature indicates that sub-ppm levels of a variety of chemicals at ranges of one kilometer or more are routine. Exhibit 3 contains detection limits and effective ranges for commonly measured chemicals.
Exhibit 3 Potential Detection Limits for Air Pollutants Using DIAL¹ |
UV/Visible DIAL System |
Pollutants |
Detection Limits² µg/m3 |
Detection Range in Meters |
Benzene (C6H6) |
10 |
800 |
Mercury (Hg) |
0.5 |
3000 |
Nitric Oxide (NO) |
5 |
500 |
Nitrogen Dioxide (NO2) |
10 |
500 |
Ozone (O3) |
5 |
2000 |
Sulfur Dioxide (SO2) |
10 |
3000 |
Toluene (C7H8) |
10 |
800 |
Xylene (C8H10) |
20 |
500 |
Infrared Dial System |
Acetylene (C2H2) |
50 |
800 |
Ethane (C2H6) |
20 |
800 |
Ethylene (C2H4) |
10 |
800 |
Higher Alkanes (CxHx) |
40 |
800 |
Hydrogen Chloride (HCI) |
20 |
1000 |
Methane (CH4) |
50 |
1000 |
Methanol (CH3OH) |
200 |
500 |
Nitrous Oxide (N2O) |
100 |
800 |
1 Values are from National Physical Laboratory, United Kingdom
2 The detection limits apply at a range of 200 m for a 50-meter plume
Oil Spill Tracking on Open Water
Yamagishi et al. (2000) report on the use of LIDAR in detecting and tracking oil spills and sheens over open water. The instrument is mounted in a small plane that overflies the area of concern. The laser emits in the ultraviolet range, which causes a fluorescence response that is captured by an imaging camera. The wavelength response strength is measured and can be analyzed to determine the type of petroleum product released. In addition, the method can also be used to estimate the thickness of the oil on water.
Remote Sensing of Chemical and Biological Warfare Plant Effluents from Aircraft
Sandia National Laboratories is developing a multispectral LIDAR system for deployment on unmanned aircraft. The system is designed to detect and image effluents that are associated with the production of chemical and biological warfare agents. A tunable UV laser is envisioned for the source with the aircraft operating at between 1 and 10 kilometers above ground surface (Hargis 1998).
Remote Sensing for Natural Gas Pipeline Leaks from Aircraft
Lenz et al. (2005) report on a Department of Energy/Department of Transportation co-sponsored demonstration of the ability of airborne DIAL LIDAR to detect gas leaking from underground pipelines. The Airborne Natural Gas Emission LIDAR (ANGEL) system operates from a fixed-wing aircraft flying between 100 and 150 mph at a height of approximately 1,000 ft. It has two DIAL lasers tuned to detect methane and ethane. The system has a scanning component that is pre-programmed with the pipeline's location data to allow it to focus the lasers on the pipeline so that the aircraft does not have to be directly over the pipeline to compensate for aircraft yaw, pitch, and roll. A digital camera records the ground surface beneath the aircraft and provides the background for superimposing the laser results. The final product is a map showing the exact location of any gas leaks and an estimate of their concentration.
A portable and less complex system was also tested at the Department of Energy/Department of Transportation co-sponsored demonstration (U.S. DOE 2004). This system, which can be mounted on the undercarriage of a helicopter, consists of a methane-detecting DIAL LIDAR, digital imaging camera, and GPS unit. The pipeline is flown over by the helicopter at 30 mph and visual observation of pipeline markers is used to guide the aircraft. The location uncertainty of the equipment used at the demonstration was �100 ft.
Both systems were generally successful in identifying leaks of 500 standard cubic feet (scf) or more and much less successful with leaks of 100 scf or less. The tested prototypes have since been modified to improve performance.
Particulate Monitoring for Ore Handling Facilities
The Institut National d'Optique (Canada) has developed and demonstrated a LIDAR system for monitoring industrial particulates. To protect the more sensitive elements of the equipment, the laser, detector, computer, and acquisitions electronics are housed in a controlled environment with the LIDAR head (optical transmitter and receiver) connected by fiber optic cables to the laser and detector. The head is mounted on a control unit that can be remotely operated to scan up to 355 degrees laterally and ±90 degrees vertically. The demonstrations were at port facilities where various ores were being unloaded and fugitive dust control was required. The system successfully imaged particulate release and was used to identify and correct loading practices to reduce emissions. The value of this system over high-volume stationary air samplers is that it gives real-time data on how far the particulates are from the source, total average release, and the location of "hotspots" (B�langer et al. 2001).
Monitoring Contaminant Fluxes from Industrial Stacks
DIAL systems can be used to measure contaminant releases from industrial stacks. These measurements, combined with an estimate of the wind speed and direction, are used to estimate contaminant flux. Since the DIAL system measures instantaneous concentrations of a single contaminant across a cross section of the plume, the accuracy of the wind speed associated with the measurement at a specific time directly affects the accuracy of the flux estimate. In an experiment at a pulp and paper mill, Weibring et al. 1998(a) compared the method of using standard wind anemometers with one using a video camera to calculate SO2 flux. The wind speed was calculated by evaluating the plume change between video frames, that could be directly related in time to measurements made by the LIDAR. Since the stack was 100 meters high, the anemometer tower gave readings that were much closer to the ground than actual stack conditions. The researchers found that these readings were often affected by buildings and the adjacent forest and therefore underestimated the flux by a considerable degree. The camera technique, which only works with a visible plume, was found to be much more accurate.
Verification and Monitoring of Cap and Cover System Integrity
Heiser and Sedlacek (2006) report on the development of a DIAL LIDAR to determine the integrity of caps and covers. For wastes capped in place or for older landfills where a leachate collection system is absent and there is no generation of methane gas, the authors propose injecting a perfluorocarbon tracer into the capped area and using the open path LIDAR to determine if any of the gas escapes. Areas of high concentration of tracer gas could be pinpointed and repairs to the cover made.
Argall, P. and R. Sica. 2002. LIDAR in the Encyclopedia of Imaging Science and Technology Ed. J.P. Hornak. John Wiley & Sons Inc., New York, Jan 2002.
Balin, I. et al. 2006. LIDAR: a significant tool for regional 3D air pollutants and PBL dynamics measurements-synthesis of a series of field results obtained in the last decade. Geophysical Research Abstracts, Vol 8, 10120.
Belanger, B., A. Fougeres, M. Talbot, and J. Cormier. 2001. Industrial site particulate pollution monitoring with an eye-safe and scanning industrial fiber LIDAR. Water, Ground, and Air Pollution Monitoring and Remediation, 6-7 November 2000. Proceedings of SPIE - The International Society for Optical Engineering, Vol 4199, pp 67-76. [abstract]
Chambers, A. 2003. Well Test Flare Plume Monitoring Phase II: DIAL Testing in Alberta. Alberta Research Council Inc.
Chambers, A. 2004. Optical Measurement Technology for Fugitive Emissions from Upstream Oil and Gas Facilities. Alberta Research Council Inc.
Chambers, A. and M. Strosher. 2006. Refinery Demonstration of Optical Technologies for Measurement of Fugitive Emissions and for Leak Detection. Alberta Research Council Inc.
Chambers, A., et al. 2006. DIAL measurements of fugitive emissions from natural gas plants and the comparison with emission factor estimates. 15th International Emission Inventory Conference: Reinventing Inventories - New Ideas in New Orleans, 16-18 May 2006, New Orleans, Louisiana. http://www.epa.gov/ttn/chief/conference/ei15/session14/chambers.pdf
Frish, M. and J. Melnyk. 1996. Detect fugitive emissions with lasers. Hydrocarbon Processing, May 31 1996. [abstract]
Frisch, L. 2003. Fugitive VOC-Emissions Measured at Oil Refineries in the Province of V�stra G�taland in South West Sweden - A Success Story: Development and Results 1986 - 2001. County Administration of V�stra G�taland, Report No. 2003:56.
Gittins, C., W. Lawrence, and W. Marinelli. 1998. A frequency agile bandpass filter for direct detection LIDAR receivers. Applied Optics, Vol 37 No 36, pp 8327-8335. [abstract]
Grant, W. 1999. Analytical methods for monitoring smokes and aerosols from forest fires: review, summary and interpretation of use of data by health agencies in emergency response planning. Health Guidelines for Vegetation Fire Events, Lima, Peru, 6-9 October 1998.
Hargis, P., A. Lang, R. Schmitt, T. Henson, J. Daniels, D. Jordan, K. Schhroder, and I. Shokair. 1998. Sandia Multispectral Airborne LIDAR for UAV Deployment. SAN098-2386C.
Heidinger, A.K. and G.L. Stephens. 2000. Molecular line absorption in a scattering atmosphere. Part II: Application to remote sensing in the O2 A band. Journal of the Atmospheric Sciences, Vol 57 No 10, pp 1615-1634. [abstract]
Heiser, J. and A. Sedlacek. 2006. Using LIDAR to measure perflurocarbon tracers for the verification and monitoring of cap and cover systems. Water, Air, and Soil Pollution, Volume 170, Numbers 1-4/ February 2006, pp 345-357.
Hovde, C. 2000. Pseudo-random tone burst modulation for active remote sensing of gases using diode lasers. Laser Applications to Chemical and Environmental Analysis, February 11-13, 2000, Santa Fe, NM. Trends in Optics and Photonics, Vol 36, pp 91-93.
Lenz, D. et al. 2005. Flight Testing of an Advanced Airborne Natural Gas Leak Detection System. U.S. Department of Energy, 84 pp.
Mayor, S. et al. 2006. REAL: 1.5 micron wavelength scanning polarization LIDAR. 23rd International Laser Radar Conference, Nara, Japan, 24-28 July 2006.
Mayor, S., S. Spuler, and B. Morley. 2005. Scanning eye-safe depolarization LIDAR at 1.54 microns and potential usefulness in bioaerosol plume detection. Proceedings of SPIE: LIDAR Remote Sensing for Environmental Monitoring IV.
Mayor, S. and S. Spuler. 2004. Raman-shifted eye-safe aerosol LIDAR. Applied Optics Vol 43 No 19, 1 July 2004, pp 3915-3924.
OConnor, S., H. Walmsley, and H. Pasley. 1998. Differential absorption LIDAR (DIAL) measurements of the mechanisms of volatile organic compound loss from external floating roofed tanks. EUROPTO Conference on Spectroscopic Atmospheric Environmental Monitoring Techniques, Barcelona, Spain, SPIE Vol 3493. [abstract]
Orr, B. 2001. Instrumentation for Multiplex Spectroscopic Sensing. U. S. Air Force Office of Scientific Research Report No. AOARD-99-04. [abstract]
Rairroux, P., H. Schillinger, S. Niedermeier, M. Rodriguez, F. Ronneberger, R. Sauerbrey, B. Stein, D. Waite, C. Wedekind, H. Wille, L. Wöste, and C. Ziener. 2000. Remote sensing of the atmosphere using ultrashort laser pulses. Applied Physics B 71, pp 573-580.
Sharma, S., J. Madey, E. Szarmes, and D. Tratt. 2000. Remote sensing applications of a free-electron laser LIDAR. International Geoscience and Remote Sensing Symposium 2000.
Simeonov, V., B. Lazzarotto, P. Quaglia, H. van den Bergh, and B. Calpini. 2000. Three-wavelength UV ozone DIAL based on a Raman cell filled with two Raman active gases. 20th International Laser Radar Conference. [abstract]
Singh, U.N. (ed.) 2002. LIDAR remote sensing for industry and environment monitoring. Proceedings of SPIE - The International Society for Optical Engineering, Vol 4153.
Singh, U.N. (ed.) 2002. LIDAR remote sensing for industry and environment monitoring II. Proceedings of SPIE - The International Society for Optical Engineering, Vol 4484.
Sj�holm, M., P. Weibring, H. Edner, and S. Svanberg. 2004. Atomic mercury flux monitoring using an optical parametric oscillator based LIDAR system. Optics Express, Vol 12, Issue 4, pp 551-556.
Soriano, C., F. Rocadenbosch, C. Puente, A. Rodriguez, J. Baldasano, and A. Comerón. 1998. Confirmation of a multilayer arrangement of aerosols in the Barcelona air basin using two independent LIDAR systems. Spectroscopic Atmospheric Environmental Monitoring Techniques, Proceedings of SPIE, Vol 3493, pp 222. [abstract]
Spuler, S. and S. Mayor. 2006. High-energy multipass forward Raman shifter as an eye-safe laser source for LIDAR. 23rd International Laser Radar Conference, Nara, Japan, 24-28 July 2006.
Spuler, S. and S. Mayor. 2006. Scanning eye-safe elastic backscatter LIDAR at 1.54 µm. Journal of Atmospheric and Oceanic Technology, Vol 22, pp 696-703.
Stelmaszczyk, K., A. Czyzewski, A. Szymanski, A. Pietruczuk, S. Chudzynski, K. Ernst, and T. Stacewicz. 2000. New method of elaboration of the LIDAR signal. Applied Physics B Lasers and Optics, Vol 70 No 2, pp 295-299. [abstract]
Uddin, W. 2001. Remote Sensing Laser Measurements of Air Pollution, Year 1 Study in North Mississippi. Center for Advanced Infrastructure Technology, University of Mississippi.
U.S. DOE. 2004. Field Testing of Remote Sensor Gas Leak Detection Systems. Rocky Mountain Oilfield Testing Center (RMOTC), Casper, WY. 261 pp.
U.S. Geological Survey. Center for LIDAR Information, Coordination, and Knowledge.
von Zahn, U., G. von Cossart, J. Fiedler, K.H. Fricke, G. Nelke, G. Baumgarten, D. Rees, A. Hauchecorne, and K. Adolfsen. 2000. The ALOMAR Rayleigh/Mie/Raman LIDAR: objectives, configuration, and performance. Annales Geophysicae, Vol 18 No 7, pp 815-833. [abstract]
Wamsley, P.R., C.S. Weimer, J.T. Applegate, S.P. Beaton, and B.S. Beyer. 1999. Mobile system for open-path trace gas detection in the mid-infrared using a Raman-shifted Cr:LiSAF source. Application of LIDAR to Current Atmospheric Topics III, 22 July 1999, Denver, CO. Proceedings of SPIE - The International Society for Optical Engineering, Vol 3757, pp 142-150. [abstract]
Wamsley, H., and S. OConner. 1998. The accuracy and sensitivity of infrared differential absorption LIDAR measurements of hydrocarbon emissions from process units. Pure Appl. Opt., pp 907-925.
Wayson, R., Gregg G. Fleming and Brian Kim. 2001.
The Use of Light Detection And Ranging (LIDAR) for Determining Plume Characteristics. Progress Report, Report No: FAA-AEE. U.S. DOT, Research and Special Programs Admin., John A. Volpe National Transportation Systems Center, Air Quality Facility, Cambridge, MA, 7 pp.
Webb, J., R. Spellicy, and R. Brewer. 2006. Using FTIR spectrometry for industrial and environmental gas analysis. EM-Pittsburgh, Air and Waste Management Association.
Weibring, P., M. Anderson, H. Edner, and S. Svanberg. 1998(a). Remote monitoring of industrial emissions by combination of LIDAR and plume velocity measurements. Applied Physics B 66, pp 383-388. [abstract]
Weibring, P., H. Edner, S. Svanberg, G. Cecchi, L. Pantani, R. Ferrara, and T. Caltabiano. 1998(b). Monitoring of volcanic sulphur dioxide emissions using differential absorption LIDAR (DIAL), differential optical absorption spectroscopy (DOAS), and correlation spectroscopy (COSPEC). Applied Physics B Lasers and Optics, Vol 67 No 4, pp 419-426. [abstract]
Weidauer D., K.H. Eickel, W. Lahmann, L. Nikowa, M. Ulbricht, K. Weber, C. Weitkamp, and J.P. Wolf. 1998. Standardization of DAS LIDAR measurements. Proceedings of International Laser Radar Conference, 1998.
Wetjen, E., M. Allen, W. Marinelli, C. Gittins, C. Gmachl, F. Capasso, A. Hutchinson, D. Sivco, J. Baillargeon, and A. Cho. 2000. Pseudo-random code-based differential absorption LIDAR in the LWIR spectral region. Laser Applications to Chemical and Environmental Analysis, February 11-13, 2000 Santa Fe, NM. Trends in Optics and Photonics, Vol 36, pp 74-76.
Yamagishi, S., K. Hitomi, H. Yamanouchi, T. Yamaguchi, and T. Shibata. 2000. Scientific approach to improve slicks monitoring using a fluorescence LIDAR. From the Nakhodka to the Erika: Exchange of Experience in At-Sea Response to Offshore Oil Spills by Passing Ships, Brest, France 6-7 July 2000. pp 77-85.
Zhang, K. and H. C. Frey. 2006. Road grade estimation for on-road vehicle emissions modeling using light detection and ranging data. Journal of Air and Waste Management Association, June 2006.